SCIENTIFIC METHOD / SCIENCE & EXPLORATION
Science inches closer to real BioShock-style plasmids
No, not Electro Bolt. But human genetic augmentation is a lot closer than you think.
by Michael Westgarth Feb 12 2014
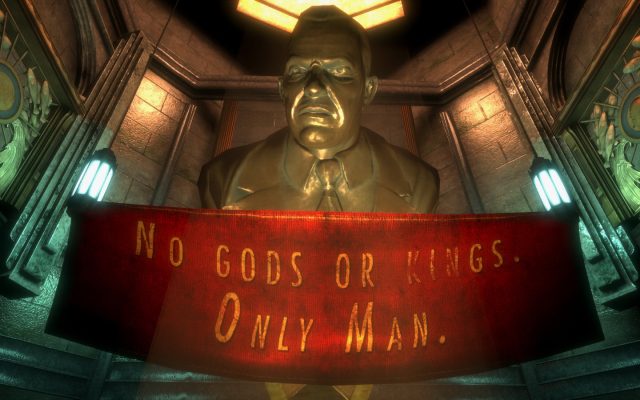
In 1953, molecular biologists James Watson and Francis Crick released what would be one of the most groundbreaking scientific discoveries of the modern age. The publication of their research paper Molecular Structure of Nucleic Acids: A Structure for Deoxyribose Nucleic Acid in the scientific journal Nature marked the end of a heated race to find the molecular structure of DNA, marking the dawn of molecular genetics. But neither man (nor their then-uncredited co-discoverers Rosalind Franklin and Raymond Gosling) could have envisioned how their work would permeate into every facet of modern society, including pop culture.
“No gods or kings. Only man.”
These are the words that greeted players to the underwater city of Rapture in the 2007 video gameBioShock. Founded on the objectivist philosophies of entrepreneur Andrew Ryan, the city was intended as a haven for the world’s greatest free-thinking minds. Free from the “oppressions” of politics, religion, and society, the citizens of Rapture could indulge in their artistic, scientific, and commercial pursuits.
The absence of ethical, legal, and sociological barriers within Rapture, combined with the discovery of the infamous genetic manipulator ADAM, resulted in the premature birth and prolific advancement of molecular genetics. The ADAM serum—a stem-cell-rich excretion of a previously undocumented sea slug—had the uncanny ability to replace or regenerate human cells and tissues.
Above sea level, the discovery of a panacea such as ADAM could have ushered in a new era of medical wonderment. Rapture’s libertarian founding philosophies, however, led to the development of a range of super power-granting “plasmids.” With the right plasmids and enough ADAM, players and citizens alike could wield lightning, fire, and ice through their fingertips, use telekinesis and hypnosis, and even turn invisible.
The upcoming BioShock Infinite downloadable content, titled Burial at Sea Episode Two, will offer players a chance to return to Rapture during the heyday of heavy plasmid use, and it will likely re-captivate many players with the fanciful idea of externally administered genetic “power-ups.” But the ideas behind this scenario aren’t entirely confined to the realm of science fiction. In fact, the latest scientific literature suggests that human genetic modification through real-life plasmids may be possible in the near future.
Non-BioShock plasmids
In real life, plasmids are small loops of DNA found within bacteria, existing independently of the core bacterial chromosome and containing far fewer genes. As with BioShock‘s plasmids, different bacterial plasmids grant different traits to their bearers and can be passed from organism to organism via a process called horizontal gene transfer.
To clarify, genes transferred vertically are passed from one generation to the next—from parent to child. Genes transferred horizontally can be passed between organisms of differing generations and even species. This act of cross-organism gene transfer wasn’t thought possible prior to the work of American molecular biologists Edward Tatum and Joshua Lederberg in the 1940s.
Tatum and Lederberg’s discovery was made following observations of a mixed culture of two E. colistrains with complementary metabolic deficiencies. In these cultures, a third strain, capable of surviving without nutrient supplements, would arise. It was known at that time that E. coli did not mate sexually—like all bacteria, it reproduces asexually, dividing into like-for-like copies through binary fission. But Tatum and Lederberg hypothesized that the third strain came about because genetic material was being “shared” on contact.
The two biologists also proposed that there was a “fertility factor” that allowed for the genetic exchange. But it wasn’t until the dawn of molecular genetics that the fertility factor was found to be a circular loop of DNA that existed independently of the bacterial genome—a plasmid.
Renamed the F-plasmid, the short sequence of DNA contains all the genes necessary for a bacterium to form a pilus—a long, thin, tentacle-like protrusion—and attach itself to another bacterium lacking F-plasmid. The pilus reels in the donor bacteria, and a copy of the F-plasmid, along with the bacterial chromosome, is transferred into it horizontally. This process is known as conjugation.
But conjugation is not F-plasmid’s only trick. Infrequent cellular mishaps can cause an F-plasmid to accidentally integrate into the main bacterial chromosome. If and when it’s excised by the cell, the F-plasmid often takes adjacent DNA along with it, including entire genes. F-plasmid derivatives known as R-factors, for example, contain an additional suite of antibiotic resistance genes. Other transferable traits could include toxin production, viral resistance, enhanced longevity, and more.
As interesting as the plasmids themselves are, what’s more interesting is how they can be manipulated for use in genetic research. By artificially inserting non-bacterial genes into an F-plasmid and introducing them to a bacterial culture, the foreign genes can be amplified through horizontal and vertical transfer, and the gene can be studied in a simple bacterial environment.
These artificial bacterial plasmids are now routinely used for the study of bacterial and non-bacterial gene function and the cloning and sequencing of DNA—the Human Genome Project relied on them. Genetically modified bacteria also offer a cheap and efficient source of human insulin, crop pesticides, and soil fertilizers. These have been used industrially for decades.
One genus of bacteria, Agrobacterium, takes conjugation one step further by horizontally transferring plasmids directly into the genome of plants. Agrobacteria were well-known to researchers in both agriculture and science, who have studied them for over 100 years because they trigger crown-gall disease—a plant ailment that results in the growth of root tumors.
But it wasn’t until the 1940s that Armin Braun, of the then-Rockefeller Institute for Medical Research, suggested the transfer of a genetic “tumor inducing principle” between Agrobacterium and plant. At the time, Braun’s idea came from his observation that the aforementioned plant tumors did not contain a significant volume of Agrobacterium. Now, the molecular basis of Braun’s elusive principle is known as the Ti (tumor inducing) plasmid.
Upon detection of an open plant wound, Agrobacteria attach themselves to an internal plant cell and inject a copy of the Ti-plasmid into it. The Ti-plasmid genes are then read by the host cell’s cellular machinery, which is instructed to integrate Ti-plasmid genes directly into the plant genome.
From there, the host cell reads the Ti-plasmid genes, which include various plant growth hormones, resulting in rapid plant cell proliferation and the formation of tumors—tumors that, under instruction from the integrated Ti-plasmid, produce nitrogen-rich molecules as a food source for the surroundingAgrobacteria.
As a proficient genetic hacker, Agrobacteria—and the species Agrobacterium tumefaciens in particular—have become the plant biologist’s tool of choice. Just like the aforementioned F-plasmid modifications, the Ti-plasmid can be altered to include any gene of interest. It’s this technology and others like it that have led to the creation of genetically modified plant seeds with added pesticide production, salt tolerance, nutritional value, and more, such as The Golden Rice Project.
The intrigue surrounding Agrobacteria doesn’t even stop there. More recent studies have shown that under strict laboratory conditions, Agrobacteria can be encouraged to transmit Ti-plasmid into yeast cells, fungal cells, algae cells, and even cultured human cells.
Still, for better or worse, on-demand, bacterial-plasmid-mediated alterations of entire human genomes is nothing but science fiction with current technologies. But a real-world equivalent to BioShock‘s plasmids may lie elsewhere—the simple yet powerful virus.
Viral for good
Nature’s true minimalists, viruses are often described as being at the “edge of life.” Consisting only of a small amount of genetic material encased in a protein coat—and an additional bubble of oil if it’s particularly fancy—viruses do not eat, breathe, or excrete any exogenous matter whatsoever. Virusesare, however, expert genetic hackers that exist for one simple purpose—replication. Viral genetic material, like bacterial plasmids, is incredibly succinct, containing only the genes necessary for this purpose.
After a virus gains entry to a cell—human or otherwise—the viral coating breaks down, leaving its genetic material exposed. In many cases, these viral genes program the host cell to assemble new viruses until the cell quite literally bursts. But there’s a group of animal-infecting viruses called retroviruses that like to do things a bit differently. Instead of manipulating host cells to construct new viruses upon infection, retroviruses integrate their genetic material directly into the host’s genome, where it can lie dormant for many years before becoming active—as is the case with HIV.
On rare occasions, retroviruses infect germline cells—which can, on even rarer occasions, give rise to offspring containing a copy of the retroviral DNA within every cell of its body. It’s at this point that the virus becomes what is known as an endogenous retrovirus (ERV). Although our ancestors evolved systems to deactivate most ERV genes, history shows that we’re not half as good at removingthem from our genomes. As such, current estimates put ERVs at five to eight percent of our total genomic DNA, a significant proportion of which have been present in our evolutionary timeline for millions of years.
Their simplicity, combined with the relative ease with which they enter cells and control the cells’ DNA (or, in the case of retroviruses, permanently alter their DNA) has made viruses an extremely useful tool in genetic research. For example, researchers can replace virus genes with a gene of scientific interest, creating a virus that can safely and efficiently transform cell cultures. The cell specificity of many viruses also allows scientists to target a specific tissue of a particular species—a trait that has been exploited in gene therapy.
Despite their scientific utility, discussions continue to this day over whether viruses deserve a place within the realm of living organisms. It may be surprising to some that inheritance—a basic feature of the living—is exhibited in genetic entities even simpler than viruses.
Transposable elements, or “transposons,” are short lengths of DNA that are able to move from one location in a genome to another. Unlike a virus, however, transposons never leave the cell they started in. Like ERVs, transposons have inhabited our DNA for millions of years, and parts of transposons comprise almost a half of the human genome. This makes transposons (and ERVs) ideal for tracing back evolutionary lines and identifying speciation events.
Each of us contains within our genomes thousands of different transposons—the vast majority of which have been rendered inactive by mutations—but active transposons can still spell trouble for our cells.
Transposons are often mutagenic: they can turn a gene off by inserting themselves into it, reactivate a gene by exiting it, or alter a gene’s rate of expression by inserting themselves into regulatory sequences. Additionally, transposons can leave irreparable gaps in their wake, cause DNA repeats to build up, and even form new and unwanted genes. In most cases, the problems caused by transposons will only affect the DNA of individual cells—typically, the DNA is repaired by the cell or, failing that, can result in programmed cell death. Even so, transposons have been implicated in anumber of diseases, including haemophilia, cystic fibrosis, Apert syndrome, various cancers, and more.
Although these selfish DNA parasites impart no obvious benefits to their multicellular hosts, the bacteria-transposon relationship is much stronger. Many bacterial transposons carry antibiotic resistance genes and other advantageous traits between a bacterium’s genome and its plasmids, hastening the spread of these traits throughout a population. Just like the aforementioned bacterial F-plasmid and Ti-plasmid, the exploitation of transposons’ unique abilities by scientists has offered another excellent genetic tool.
For example, a transposon containing an easily identifiable genetic sequence can be introduced into a bacterial colony. The colony can then be monitored for any noteworthy characteristics, such as altered metabolism, which is caused by the random insertion of the transposon into a bacterial genome—a process known as insertional mutagenesis. Once isolated, the bacteria’s genome can be scanned to locate the unique transposon sequence, thus revealing its location and thereby the location of the gene responsible for the interesting characteristic.
Taking the process one step further, a transposon can be modified to contain a marker gene such asGFP (green fluorescent protein). This simplifies the identification and isolation of engineered bacterial genomes, as cells containing the transposon will glow green under ultraviolet light. Similar techniques are also used to perform transposon insertional mutagenesis screens for genes in fruit flies, zebrafish, rice plants, mice, and even cultured human cells.
Knowing all this, the idea of BioShock-style plasmids seems a bit less far-fetched. Let’s say for a moment that the world’s greatest scientific minds somehow concocted a reliable transposon vector for use with live, fully grown humans. Let’s then say that multiple different concoctions were created, each featuring a different fantastical transposon that itself contained a weird and wonderful gene unlike anything seen on this Earth. Let’s go one step further and say that these serums were produced en masse for exclusive use by the citizens of an intellectually unbounded underwater city…
You get the (hypothetically plausible) picture.
Again, these plasmids aren’t possible yet.
Synthetic success
That’s not to say that customized transposons are limited to the hypothetical. A synthetic “Sleeping Beauty” transposon system is already set to be the world’s most inexpensive form of gene therapy. But transposons have size limitations that, more than anything, reduce their usefulness in medicine.
Contrary to popular belief, a gene is not a single, autonomous unit. Instead, it’s a group of distinct “modules” functioning as one. For example, all genes are proceeded by a “promoter” sequence that allows the gene to be read by the cell. Promoters are commonly joined to activator and repressor sites, allowing the rate of gene expression to be controlled. Many genes in plants and animals also contain numerous sections that can be read or skipped by the cell in a process known as alternative splicing, where one gene can contain the code for more than one protein. To make matters even more complicated, regulatory DNA sequences can be responsible for the expression of several adjacent genes—in bacteria, this unit is known as an operon.
Transposons are useful when working with relatively small pieces of DNA, but as of now they’re incapable of hosting large regions of the human genome, which may include complex mixtures of protein-coding sequences and regulatory DNA.
Both plasmids and viruses, while extremely powerful tools in research and gene therapy, also suffer from the same size limitations as transposons, and they have issues transferring multiple copies of the same gene. Additionally, all three methods result in DNA insertion at random places in the host genome, with potentially unwanted results. That’s why the possibility of efficiently and stably transferring enough genetic material into humans to allow for traits even remotely similar to those imparted by BioShock’s plasmids is currently a simple fantasy.
But the possibility may not be a fantasy forever. The next logical step in addressing these issues would be the creation of synthetic DNA constructs that can be introduced into human cells. These constructs could contain masses of DNA and exist independently from the rest of the genome—a human artificial chromosome (HAC).
Efforts to chemically synthesize entire plasmids and chromosomes are nothing new. But recent advancements in the rapidly expanding field of “synthetic biology” have yielded some truly remarkable results.
In 2006, the J. Craig Venter Institute—founded by world-renowned geneticist Craig Venter—patented what his team named the minimal bacterial genome. This electronically stored genome contained what Venter’s team concluded was the absolute minimum genetic code required for a bacterium to survive and propagate. Its purpose? To eventually act as the metaphorical backbone to the first partially synthetic bacterial species, Mycoplasma laboratorium.
The Venter Institute reached another milestone in 2010 when Science published a paper describing the first transplant of a completely synthetic genome into a “blank” bacteria. The chemically synthesized genome originated from an electronically stored copy of the Mycoplasma mycoides genome sequence. The whole genome—which in bacteria consists of a single, circular chromosome—was then inserted into an inactive and DNA-less Mycoplasma capricolum, a close relative to M. mycoides. The resulting organism was completely viable and successfully replicated into billions of genetically identical offspring.
A collaboration between American, British, Chinese, and Indian laboratories is attempting to take this technology one step further. The Synthetic Yeast 2.0 project, based at the Johns Hopkins School of Medicine, aims to chemically synthesize a minimalistic genome for insertion into a “blank” household yeast, Saccharomyces cerevisiae.
It’s important to note that yeast cells are fungal, not bacterial. They are much larger and have a complex cellular structure similar to humans and contain numerous distinct chromosomes—16 to be precise.
The success of Synthetic Yeast 2.0 would open the door to other customized yeast genomes. Used at an industrial scale, these made-to-order yeasts could produce vaccines, biofuels, and other biochemicals that cannot be synthesized by bacteria. Additionally, this yeast could contain particular sections of the human genome for a steady supply of naturally produced human proteins.
The potential applications of Synthetic Yeast 2.0 are so great that the British government has recently granted £1 million (more than $1.5 million) to Imperial College London to synthesize yeast chromosome number 11. And that’s separate from the approximately £60 million (more than $98 million) devoted to several new synthetic biology research centers and a new innovation center to help unite research and industry commercialization.
Gene alterations
How do these scientific advancements in yeast relate to human genetic augmentation? Well, it stands to reason that if artificial chromosomes can be introduced into a yeast cell, the same process could theoretically be performed with human cells.
In July of this year, The Independent reported on unpublished work by the US National Cancer Institute (NCI) claiming that a research team successfully synthesized, from scratch, the first human artificial chromosome (HAC). Not only that, but the HAC had been inserted into a strain of genetically engineered lab mice, where it remained stable without disturbing the function of the murine chromosomes.
Needless to say, the implications of the NCI’s work will be momentous. Sections of the US government have already turned their gaze to the future of HACs, with DARPA issuing a public solicitation that will award a substantial grant to any laboratory willing to research new and improved HAC methodologies.
But NCI researcher Dr. Natalay Kouprina has already envisioned the next step for HAC development—a new generation of powerful, tailor-made therapies to replace genes that have suffered damage and carry mutations that lead to disease.
“The purpose of developing the human artificial chromosome project is to create a shuttle vector for gene delivery into human cells to study gene function in human cells,” Kouprina told The Independent. “The idea is to take skin cells from a patient, turn them into stem cells, and insert HACs into these stem cells with healthy copies of the disease gene. These cells, with the extra chromosome, can then be inserted back into the patient to treat the illness.”
Major strides in HAC development have also been made by the Institute of Regenerative Medicine and Biofunction of Tottori University, Japan. The institute has published a steady stream of findings since the start of 2013 regarding its human artificial “mini-chromosomes”—constructs created by cutting away full-size human chromosomes or by joining small chunks together. It’s also working on efficient systems with which these can be transferred to mice.
For example, one successful experiment involved fully grown mice containing mini-HACs with a multi-gene region of the human genome. The region contains members of the CYP3A family of human proteins, which metabolize roughly half of all modern drugs. Mice containing the human version of CYP3A would offer an exceptionally useful model for testing new human drugs.
A similar experiment was performed with type A haemophiliac murine stem cells. The culture of cells was introduced to mini-HACs containing genes FVIII and PF4—human genes responsible for blood coagulation that are faulty in type A haemophiliacs. The experiment was successful, resulting in murine stem cells where haemophilia was partially counterbalanced by the expressed FVIII and PF4 genes.
All told, the story of molecular genetics, from its founding in the 1950s to present-day research into gene therapies and HACs, is a tale that’s even more fascinating than any of the genetic possibilities offered by BioShock. Genetic technologies are not only becoming more advanced as the years go by, they’re also getting an awful lot cheaper.
It’s unlikely that BioShock fans will be getting their hands on Incinerate or Electro Bolt any time soon, but groundbreaking HAC and stem cell research, combined with the ever-advancing synthetic genome technologies typified by the J. Craig Venter Institute and the Synthetic Yeast 2.0 project, may mark the beginning of a new era of human genetic augmentation.